Tiny Modular Reactors - what can we learn from them?
The IAEA released their 2022 version of the SMR booklet, which tracks the development of various designs. For energy hobbyists like me, it is a real treasure trove.
The booklet, “Advances in Small Modular Reactor Technology Developments, 2022 Edition” weighs in at 90MB.
Three quite tiny designs have caught my eye: the 20MWe OPEN20, the academic version of Last Energy’s approach towards nuclear, the Swiss “STAR Energy” pressure tube reactor and Moltex’s FLEX reactor. Why are these reactors interesting?
Let’s first look at the downsides of such reactors. Generally, they will require more material including - nuclear material - per unit of power. Operating expenses have to by divided by a far smaller power capacity, which typically increases generation costs per unit of energy. They do not profit form economics of “vertical” scale. In short, everything else being the same, we should expect these reactors to produce more expensive electricity.
The reason that these designs are nevertheless interesting is that the absolute amount of capital per unit is rather small and far more investors and localities are capable of investing. When the community can make a sovereign decision for an investment and is the direct beneficiary, resistance to siting should be far smaller. At least that seems to be one of the lessons of the renewable power build out.
A smaller size should also make it possible - and of course necessary - to place these reactors at far more sites, because for example the existence of a large body of water for cooling or the proximity of sufficiently sized grids are not constraining factors. Given that the safety case is often great and even in worst case scenarios the absolute amount of nuclear material is small, these reactors should be able to be placed closer to population centers. This proximity and the relatively small size could mean the waste heat of such reactors could be integrated far more easily - and maybe even profitable - into district heating systems. Given that humans in general and industry in particular learns by repetition, the hope is that these reactors will attain economics of “horizontal” scale.
Of course, this is “just theory” for now. Except for one Chinese and at least some Soviet (yes, that far back in time) attempts, the usage of small reactors close enough to population centers to power district heating has not been demonstrated. Definitely not at scale.
I would really like to see this experiment happening in the real world. The financial and physical risks are really low on the society level. If it worked, it could change a lot about who builds and operates energy systems. More carbon-free, reliable power is of course also beneficial for the question of climate change, if it is cheap enough - and used often enough.
Last Energy
Last Energy is developing a scaled-down one-loop pressurized water reactor (PWR) with and output of 20MWe and the explicit design goal of avoiding innovations around fuel, reactor physics, or material science. It uses normal LEU fuel with an enrichment of less than 5%. Further, their design philosophy calls for the straightforward demonstration of risk reduction using conservative assumptions and fewer critical systems, a modular design that facilitates factory construction for accelerated delivery time and reducing required on-site activities, the minimization of siting constraints by using air-cooling as “ultimate heat sink” and having a small physical footprint.
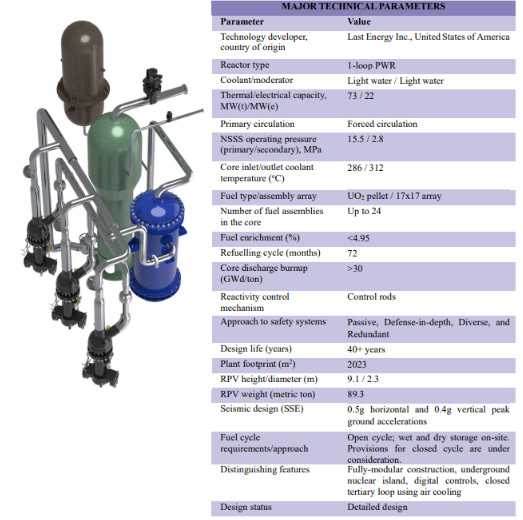
They do not opt for an integral design, but have a “traditional”, external steam generator with separates the forced reactor coolant flow from the turbine steam. The reactor coolant is pumped through the reactor by vertical, canned, single stage centrifugal pumps that operate with variable frequency drives positioned at the cool side of the steam generator. There are three reactor coolant pumps for resilience and triple redundancy.
The reactor features a passive decay heat removal and emergency core cooling system to protect the system integrity during unlikely accident scenarios. Last Energy will contain the nuclear system inside a subterranean, modular, leak tight, steel enclosure, that prevents any inadvertent release of radionuclides during either normal operating or accident conditions.
The operating concept envisions a largely autonomous operation with on-site operators having access to only critical process information and alarms. The reactor is operated on a 72-month fuel cycle.
Last Energy’s aggressive timeline calls for 10 completed reactors by 2026 and the have a MoU with a Polish special economic zone.
STAR
STAR is the “Safe, Tiny, Atomic Reactor” developed by Star Energy. It is a 30MWth, 10MWe light water reactor that features an uncommon layout, wherein the coolant of a double-loop primary loop circulates through individual pressurized channels instead of an outer pressure vessel. It uses HALEU at 19% enrichment to further shrink the dimensions of the reactor. The designer claim that the whole system will weigh only 20t. If this were true, the whole system could be assembled in a factory and be transported to the installation site by land, sea and even air.
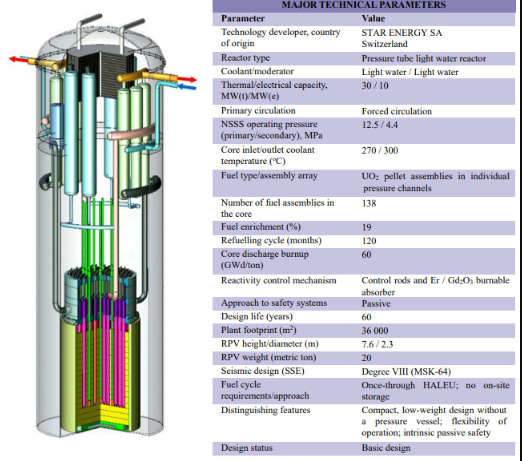
The reactor core consists of 138 pressure channels, which are made out of an outer tube welded into the lower plate of the primary coolant inlet chamber and an inner tube welded into the lower plate of the primary coolant outlet chamber. The coolant is fed to the core through the primary coolant inlet chamber, from where it flows along the outer section of a pressure channel and rises along the inner tube. The fuel assemblies are inside the inner tube. The tubes are made from a zirconium-niobium alloy, and the space between the tubes is supposed to be filled with neutron-transparent material. The reactor core is encased by a neutron reflector. The fuel cycle length is set to 10 years. During normal operation, no boric acid is supposed to be used for reactivity control; it could be used as an emergency shut down system, though.
Emergency reactor cooling is provided by pressurized water from an emergency tank into the reactor vessel. Long-term cooling system is designed to passively remove decay heat from the reactor through steam generators and natural circulation of coolant even if both circulation pumps failed. An additional steam condenser installed at the top of the reactor vessel defends against LOCA events. The designers estimate a LCOE of 2.8 cents / kWh. Personally, I think that seems a tad too optimistic.
Moltex
Another addition is the 16MWe, 40MWth graphite-moderated molten-salt reactor FLEX developed by MoltexFlex, which is a subsidiary of Moltex. It is supposed to operate at 700° C and features completely passive cooling and reactivity control. Moltex gives an LCOE estimate of $US44/MWhe.
This design is the second implementation of the “Stable Salt Reactor” general design idea. The defining characteristic of these reactors is that the fuel salt is contained inside vented fuel tubes. A different salt free of fission products or nuclear material surrounds these fuel tubes as coolant. The first SSR is the “SSR-W”, a fast reactor that is capable of burning already used nuclear fuel. FLEX is operating on 6% enriched uranium in the thermal spectrum. In FLEX, there are no coolant pumps. In fact, there are no moving parts at all involved in the circulation of the coolant. It operates passively due to heat-induced differences in coolant density.
The tube material needs to be chemically stable and resistant to neutron damage. Moltex has chosen the material of the UK’s Advanced Gas-Cooled Reactors for this. It will probably be quite a challenge to demonstrate the corrosion is not an issue despite the flouride chemistry for the fuel cycle length of 20 years. Because the nuclear material is not moved during operation, accounting of the nuclear inventory by regulating bodies like the IAEA should be far easier than for other molten-salt designs, where the fuel salt in pumped through the core during normal operations.
A new invention that is supposed to be deployed with this reactor is a liquid neutron absorber in a kind of “inverted thermometer”. The “bulb” of the “thermometer” is placed at the top of the reactive zone, where the coolant salt is hottest. The “stem” extends down into the graphite moderator. When the temperature rises above anticipated levels, the liquid expands into the reactive zone to quell reactions.
“Emergency” cooling is “on” constantly. That means that the reactor will “lose” 0.5% of its thermal power via passive air cooling. This is enough to transport all decay heat into the environment and stabilize the reactor in all scenarios. Reactivity is further controlled by burnable poison in the graphite and more poison can be added to the coolant salt, if that appears to be necessary.
Moltex claims that the safety case of the reactor does not depend on the primary coolant loop, because the reactor can cope with a loss of all cooling via that route. That means that heat production and power conversion are separated. This should simplify the safety case considerably. Multiple of these reactors could be deployed to a larger thermal energy storage system, which could then be used to power a larger, load-following turbine.
An advantage of this design is supposed to be that all major tasks for operators are done autonomously by the design via passive mechanisms. This should lead to a reduction of operator interventions; which seems a necessary given its small power output and the resulting effect of labor costs on LCOE.